Summary
This is the second Substack post of a series of posts describing how vapes work, the aerosol they generate, their properties, their optimal regime of operation, overheating conditions and dry puffs, as well as comparisons with tobacco smoke and other aerosols. Post 1 Understanding vape aerosols can be found in this link
Understanding how vape aerosols form, operate and can be tested provides the knowledge to understand their pleasurable usage, their toxicity profile and relative safety with respect to tobacco smoke and other aerosols and pollutants.
This knowledge (which i try to present accessibly) reassures our confidence on the role of vapes in harm reduction and serves us to counter ignorant and malicious disinformation.
Post 2:
I described vape emissions in Post 1 as analogous to the aerosol emerging as we boil water in a kettle. These are liquid based aerosols whose formation involves two phase changes: evaporation and condensation. Vape aerosols also involve low energetic “thermal degradation” reactions from the heating of the e-liquid, producing byproducts found in minute quantities under “normal” operating conditions.
In this post I explain in detail how these “normal” operating conditions emerge from thermal physical processes taking place while vaping, based on the trade-off between between energy released and absorbed. These conditions define an “Optimal Regime” that can be tested in the laboratory.
Physical processes in vaping
When generated through normal boiling under the appropriate “optimal” physical parameters (power, airflow, resistance, airflow, temperature), vaping is pleasurable for its users and ~ 99% of the aerosol mass in both the gaseous phase and the droplets is made of the solvents PG, VG, nicotine and water vapor, with byproducts (from the solvents and the flavor chemicals) found in minute yields.
Users are guided (often through trial and error) by their sensory perception to assess when vaping is pleasurable, thus “normal” or “optimal”. However, is there a scientific basis to determine what is an “optimal” regime in vaping? Yes, please keep reading.
The use of a vape device can be understood in terms of thermal physics (heat exchanges) as the cyclic (per puff) balance of energy exchange, consisting in the following processes that take place in every puff close to thermal equilibrium. These processes are
E-liquid flows into the cotton wick
Energy (heat) is supplied by the battery and released to the coil wires
Part of the energy is used (released) to heat by conduction the e-liquid in the wick to bring it to boiling temperature. Part is used (absorbed) to vaporize the liquid (latent heat of vaporization)
Almost simultaneously the user inhales, exerting forced convection on the e-liquid vapor (energy is absorbed) , cooling and condensing it to form the aerosol
Not all energy (heat) is used, all these processes leave (release) a residual energy contained in the atomizer, which heats the coil, the liquid, the air-vapor mixture and the walls
The residual energy increases the capillarity rate of e-liquid in the wick
They can be illustrated graphically (follow the numbers above in figures 1 and 2 below):
Since the processes do not occur under thermal equilibrium and are not 100% efficient, they dissipate energy (residual heat) at every puff cycle, but under optimal conditions the residual heat is not problematic.
Vaping parameters for laboratory testing
Vapers do not need to know physics or chemistry to assess intuitively (guided by their sensory perceptions) the fulfillment of this energy balance, often in a trial and error basis. Individual vapers perceive the need to adjust different parameters: intensity of inhalation, supplied power, coil resistance, nicotine levels.
Inhalation can be understood as an airflow rate (milliters per second). Devices are designed with narrow or wide mouthpieces to facilitate or harden the inhalation. The mouthpiece diameter controls its air resistance (ease of inspiratory suction, as with a straw ). There is a laboratory tested physical relation between air resistance of the mouthpiece and the airflow passing through it, which clearly determines the intensity of inhalation of vaping devices. This is depicted in this graph of air resistance vs airflow
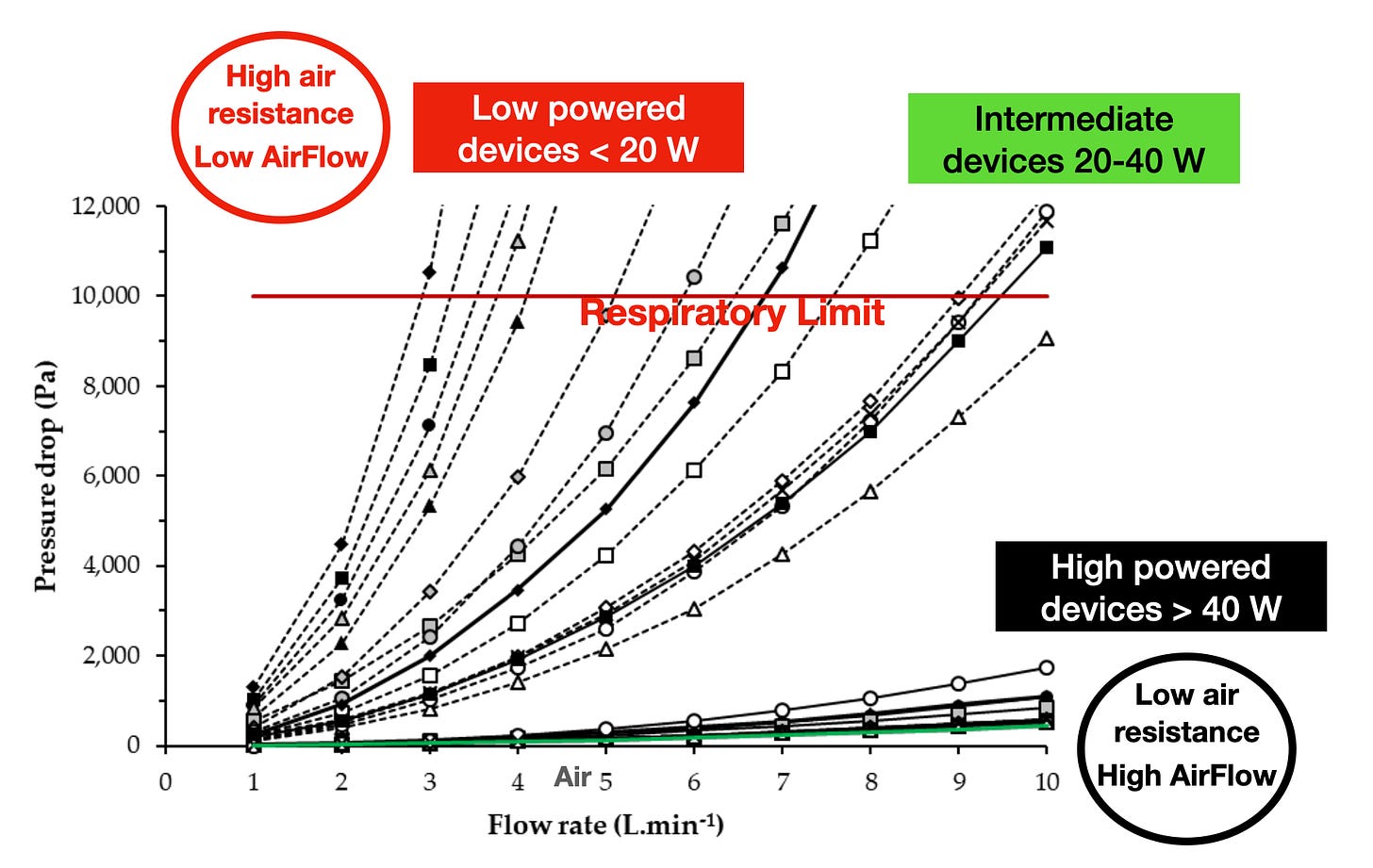
As shown in figure 2 above, low air resistance and wide mouthpieces, thus large airflow rates (around 10 L/min), facilitate vaping high powered devices (> 40 W). As a contrast, high air resistance and narrow mouthpieces, thus low airflow rates (1-2 L/min), facilitate vaping low powered devices and pods (< 20 W).
The relation air resistance vs airflow rate is perfectly consistent with the two main vape styles: “mouth to lung” (low power) and “direct to lung” (high power). Of course, this relation is not absolute for all vapers, but it clearly describes the “comfort zone” of majority use. Evidently, there is an intermediate range between high and low powered devices and low/high air resistance and airflow rate (which can also be modified with ventilation slits).
Lithium batteries used in all vapes have similar voltage V. From Ohm’s law :
Therefore for constant V we must have high coil resistance (R > 1 Ohm) goes with Low power (< 20 W) and Low coil resistance (R < 1 Ohm) with High Power (> 40 W). This is shown in the graph in figure 3:
A similar inverse relation holds between nicotine concentration and supplied power, which is proportional to puff volume.
Thus, to inhale a roughly constant nicotine mass, low power (small puff volume) go with high nicotine concentration, while high power (large puff volume) goes with low nicotine concentration. Vapers know this, as shown in figure 4 below
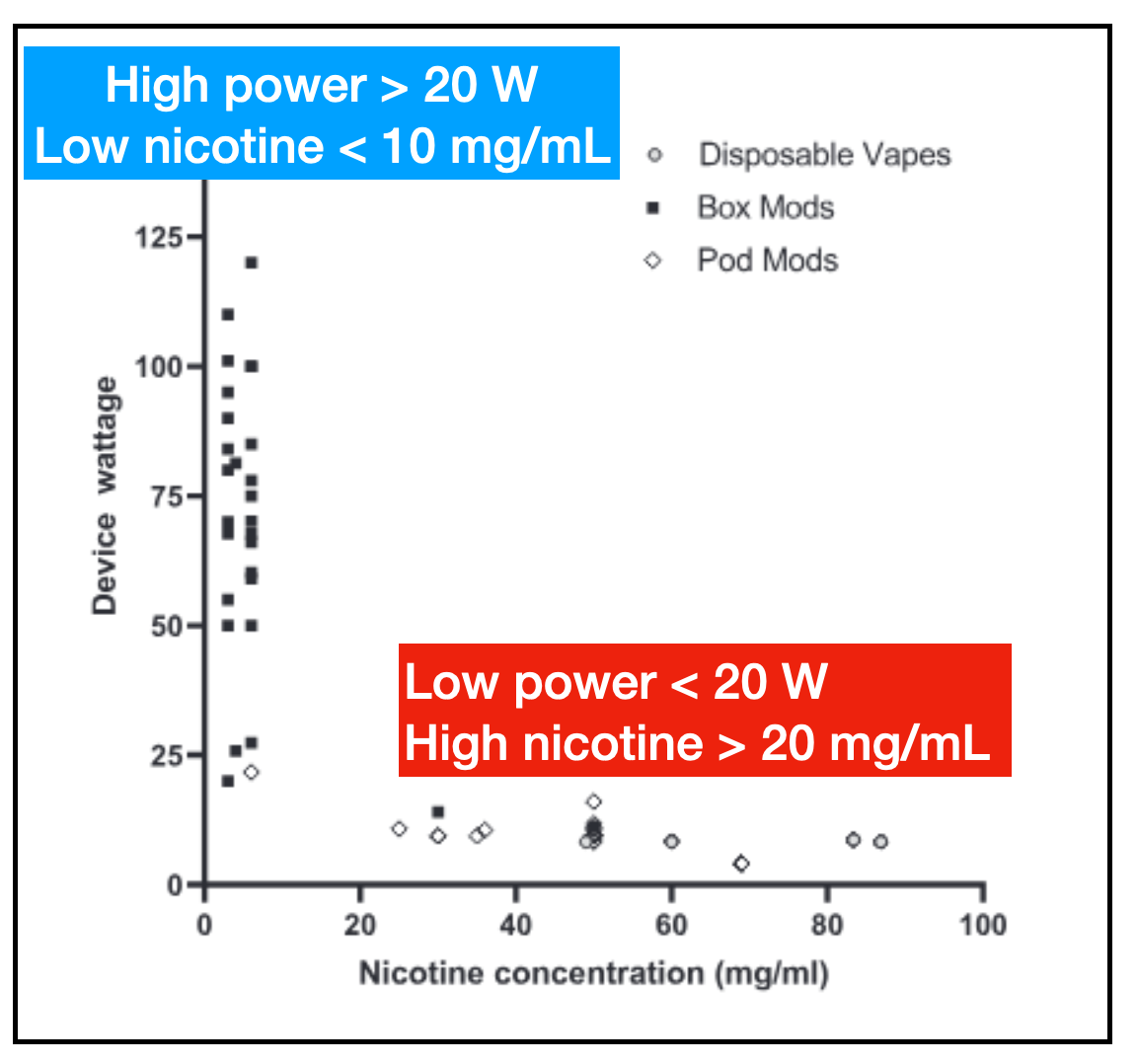
The choice of flavors and PG/VG ratio are also important factors that influence the energy trade-off and a preferred device type.
Figures 2-4 illustrate how vaping parameters understood intuitively by vapers follow from conservation laws that govern the relation between inhalation, mouthpieces, coils, supplied power and nicotine levels. Given the number of parameters associated with vaping and the wide individual variation, one wonders if a laboratory testing standard is possible. .
It is evident that consumer usage cannot be reproduced in the laboratory, since to be useful, laboratory testing requires standardization of experimental procedures that ideally should be determined from demographically dominant consumer patterns. Experiments must select a series of discrete hopefully representative values of vaping parameters. Standardization then requires conducting a series of blocks of regimented puffs (all with same puff duration and puff volume) varying different parameters to test the equilibrium between physical processes (figure 1) and considering the inter-parameter relations in figures 2-4.
Another difficulty in laboratory testing comes from the need to use “vaping machines” to simulate inhalation by pumping the aerosol from the devices, since instruments cannot be placed inside vapers’ mouths.
Evidently, no laboratory puffing protocol can reproduce consumer usage, which is far from being “regimented”. However, it is possible to conceive standardized protocols that are, at least minimally, consistent with the design and usage of different devices and diverse parameters (as we discussed before and displayed in figures 2-4).
Unfortunately, a large share of laboratory studies generating vape aerosols (to test emissions or expose in vivo and in vitro systems) do not follow a puffing standard, which makes it difficult to compare experimental outcomes. Currently all studies that use a standard refer to the protocol of CORESTA (Cooperation Centre for Scientific Research Relative to Tobacco Method 81), which is an adaptation to early vaping devices of the protocol used by the tobacco industry to test cigarettes. This protocol is roughly appropriate to test currently available low powered and pod devices, but there is a pressing need to upgrade it for testing the wide variety of vape devices in an increasing market.
Testing the optimal vaping regime in the laboratory
Under close to thermal equilibrium conditions, we expect that the mass of the inhaled aerosol should increase proportionally with each increase of power. This proportionality rate can be related to the thermodynamic efficiency of aerosol generation. It must be evaluated for each combination of device, coil, e-liquid mixture, nicotine levels and different flavors chemicals.
The mass of inhaled aerosol is difficult to measure directly, but a very accurate proxy is the mass of e-liquid vaporized MEV, which is easy to measure (by precision weighing before and after each puff). The airflow (liters per minute or mililiters per second) can be obtained by dividing puff volume by puff duration time.
The optimal vaping regime can be tested experimentally by verifying how in blocks of regimented puffs increasing supplied power W increases the mass of e-liquid vaporized MEV. The graph of W vs MEV is called the “functional curve” and its shape depends on the airflow, PG/VG mixture and nicotine levels. We can identify three heating regimes:
Optimal regime: The graph W vs MEV is a straight line: every increase of power is matched by a proportional increase of the mass MEV.
Under-heating, supplied power below the Optimal Regime is insufficient to vaporize e-liquid. The graph W vs MEV is zero or close to zero
Over-heating, supplied energy surplus increases residual heat at the expense of vaporizing e-liquid, further increasing in each residual heat and decreasing the rate of MEV generation
The functional curve displayed in figure 6 below corresponds to a Cubis device with 1 Ohm resistance, 50/50 PG mixture and 12 mg/mL nicotine. The Optima Regime is marked by a linear relation W vs MEV that extends between 7-27 W with airflow rate 1.1 L/min. The Overheating Regime extends above 27 W, as a non-linear relation W vs MEV, with MEV slowly increasing with increasing power. In the Under-heating Regime supplied power is insufficient to vaporize e-liquid and generate the aerosol.
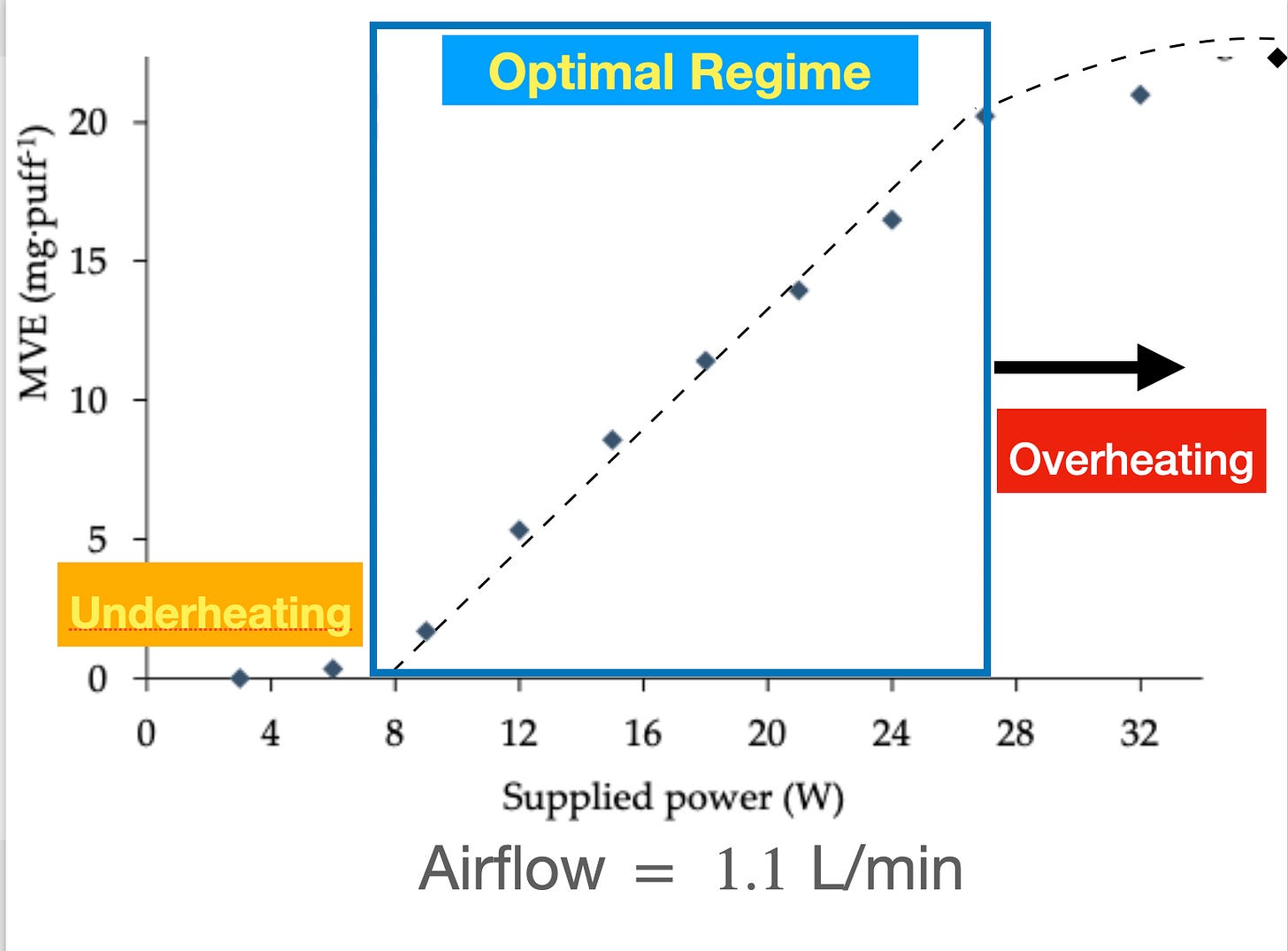
Functional curves are strongly shaped by the airflow rate, with larger airflow rates widening the power ranges of the Optimal Regime and smaller airflow rates narrowing it. The functional curve in figure 6 below shows the same Cubis device (as above) puffed at two different airflow rates: 1.1 L/min (as in the previous figure) and 10 L/min. Notice how the power range of the Optimal Regime becomes wider with increasing airflow rate (9-40 W).
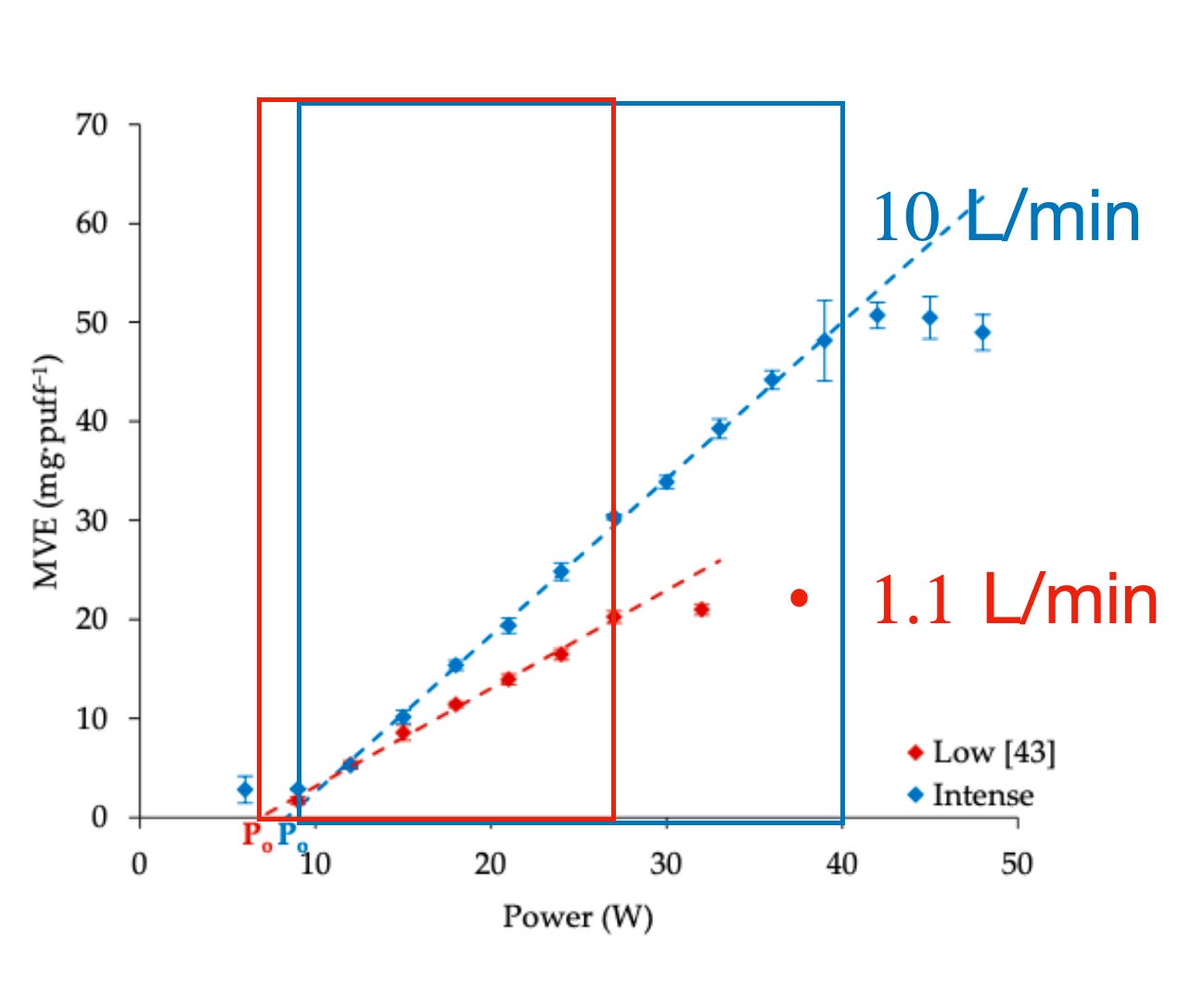
The Optimal Regime in figures 5 and 6 shows the supplied power range for a given airflow where the trade-off between the inhalation airflow (forced convection) is efficiently absorbing heat to balance the supplied heat for boiling and vaporization. The slope of the functional curve is proportional to the thermodynamic efficiency (rate of energy exchanges in figure 1).
The Optimal Regime is the power range for maximal efficiency given a choice of airflow. These are the power ranges in which a vaping device should be used, which roughly coincide with power ranges of manufacturers recommendations, though manufacturers do not disclose how they determine these ranges.
Many currently used devices allow users to fix a supplied power, coil resistance, nicotine levels and PG/VG mixture, but how can the user set up the appropriate airflow? Users fix it by controlling (often in a trial error basis) the depth and intensity of their inhalation and by opening/closing ventilation slits. The airflow rate of 1.1 L/min recommended by the CORESTA method 81 protocol is (as illustrated in figure 2) is consistent with consumer puffing low powered devices operating below 20W with the “mouth to lung” style, while high powered devices require higher airflow rates as high as 10 L/min.
The functional curves (as in figures 5 and 6) do not forbid vaping optimally a high powered sub-ohm device (> 40 W) with the CORESTA airflow, they only restrict optimal vaping with this airflow to much lower and narrower ranges of power. However, as shown in figure 2, given the wide mouthpieces and very low air resistance of these devices, it is difficult to puff them without a significantly large inhalation (airflow). A naive vaper trying to puff a high powered device with the low airflow used to puff a low powered pod will, most likely, get a hot burst of aerosol.
Temperature is often regarded as the key variable in vaping, but vaping involves many temperatures (coil, liquid, vapor, atomizer walls, mouthpiece). However, only the coil temperature matters because in normal boiling it is the same as the boiling temperature of the vapor and e-liquid around the wick (which are close to thermal equilibrium). This boiling temperature depends on the specific e-liquid mixture, not on supplied power or resistance (the effect of high supplied power is a more rapid reaching of the boiling temperature).
It is possible to determine temperatures theoretically or to measure them in the laboratory, but coil temperatures that differ from boiling temperatures are very difficult to control, even in devices sold with a “temperature control mode”. These are sub-ohm tank devices that use coils with alloys (for example nichrome) in which resistance depends on temperature. They have an electronic controller that given a coil temperature (that the user selects) will set up a variation of power and voltage to remain consistent with this temperature through Ohm’s law. However, the boiling temperature need not coincide with a coil temperature selected by the user, hence using this temperature controlled mode requires a lot of trial and error testing. Temperature values in the instrument screen are likely to be very imprecise, especially if the devices are puffed with an inappropriately low airflow.
What’s next?
We need to understand what happens when operating a vaping a device under abnormal Overheating Conditions at power levels above the Optimal Regime. Since coil temperature exceeds the normal boiling temperature of the e-liquid, as power increases this regime involves different boiling processes: nucleated boiling and film boiling, eventually leading to an end point known as “dry puff”. I will examine how several studies have produced (and continue producing) high levels of byproducts by testing the devices under conditions that produce repellent aerosols to users, but vaping machines keep operating.
This should be required reading for anyone testing these devices.